Catch them if you can
12 Sep 2016 by Evoluted New Media
When it comes to the performance of your fluorescence microscopy system, every photon is sacred – but how to catch as many as you can? Dr Martin Thomas has some answers…
When it comes to the performance of your fluorescence microscopy system, every photon is sacred – but how to catch as many as you can? Dr Martin Thomas has some answers…
In many ways optical microscopy is “old” technology, but from the current rapid pace of development in this area, one certainly wouldn't think so. So what, exactly, is going on here? The microscope, at least in the form that we would recognise, dates back at least to the days of Isaac Newton's contemporary Robert Hooke, which puts it firmly into the seventeenth century. Admittedly we had to wait a couple of hundred years more for the theoretical advances – such as those made by Seidel on the subject of optical aberrations and Abbe on the resolving power of an optical microscope – in order to put the whole field on a solid theoretical footing. But the early decades of the twentieth century arguably passed microscopy by…then, something changed.
[caption id="attachment_55453" align="alignnone" width="620"]Fluorescent molecules spend their lives playing a form of Russian roulette
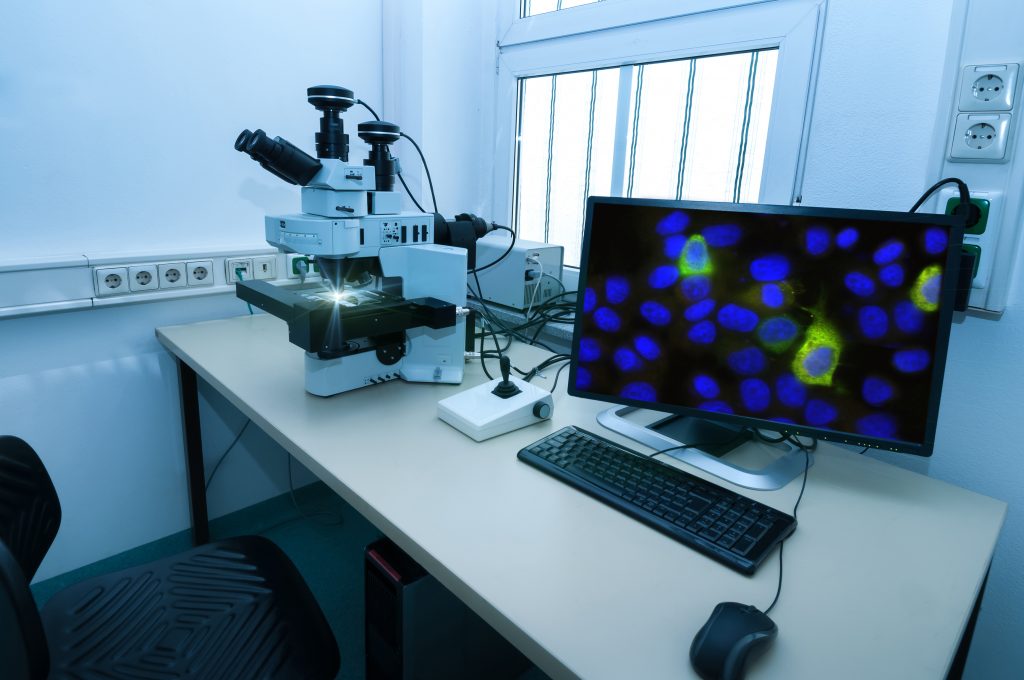
That change was the development of fluorescence microscopy techniques for the biosciences. Most biological systems, at least on the scale of sizes appropriate for optical microscopy, are pretty transparent, so to see fine structural detail one's best hope was to use highly absorbent stains that would (more or less) selectively bind to specific internal structures in order to render them visible. However, when this is done the piece of biology under study was thoroughly dead. Fluorescence is fundamentally different, and one of the reasons why it works so well as a technique is that only a small proportion of molecules display this property to a significant extent. Fluorescent molecules don't just absorb light, but they also re-emit it. Except for some unusual conditions that we'll deal with later, the emission is at a longer range of wavelengths than the absorption – which for a fluorescent molecule (fluorophore) is described as an excitation – and this allows a discrimination to be made between the two. With appropriate wavelength filtering, this allows the fluorophores to be seen against a dark background, which is a far more sensitive technique than an absorption one can ever give. Furthermore, the fluorescence properties of some fluorophores may change in a quantifiable way according to their environment, and this has been exploited to particular effect for measurement of dynamic calcium ion concentration changes in living cells by fluorescent indicators such as fura2, developed by Roger Tsien and coworkers in the 1980s.
But in all of this there is a catch. Fluorescent molecules spend their lives playing a form of Russian roulette. Every photon they absorb may well be their last, as the molecule is then in an excited state, which may either self-destruct or react with other cell constituents, giving rise to the problems of photobleaching and phototoxicity (and there may well also be other phototoxic effects resulting from photon absorption by other molecules). Even a “good” fluorophore may survive only a few tens of thousands of excitations, which makes this problem a very real one. This means that getting the best out of any fluorescence microscopy system inevitably comes down to minimising the number of excitation photons you put in, while maximising the number you get out, and the various ways in which this ratio can be improved range from the mundane to the esoteric. While the more esoteric ones (…I'm thinking two-photon here) may only be available to the well-funded few, the more mundane ones are available to everyone, so it's the discussion of these that will form the major part of this article.However, a much more attractive possibility for dealing with the lack of sufficiently short-wavelength sources has recently appeared which is instead to shift the spectral range of the fluorophore to rather longer wavelengths
Control of excitation Let's start with the input side. It has just so happened that some of the more important fluorescent indicator applications have utilised fluorophores that require excitation in the near ultraviolet (calcium measurement with fura2 again refers). For such applications this has limited the choice of light source to arc lamps such as mercury or xenon, both of which emit in this wavelength range, the former being substantially brighter at some wavelengths, but with the latter having a much more even spectrum. However, the spectral range sent to the sample must of course be limited to that of the excitation spectrum of the indicator. This can be done by a variety of methods such as optical filters (of which more later), or more sophisticated and potentially rapidly-variable devices such as a monochromator. The results are generally “bright enough”, but not so bright as to cause instant photobleaching, so for some of the tricks that we're going to be talking about it would be nice to have a few more excitation photons in hand. Also for those tricks we're going to need some fast switching, which for arc sources would have to be done by mechanical shuttering, and which would struggle to be fast enough.
For the fast switching applications, solid-state light-sources such as LEDs and (increasingly) laser diodes are much more suitable, as they can generally be switched on or off on a sub-microsecond timescale, and the additional light source electronics required in order to do this is trivial. Only in recent years have LEDs become bright enough to rival arc lamp sources, but they also have the potential advantage that you're starting with a more limited spectral range, with most ranges through the optical spectrum being pretty well represented, so there is potentially good coverage overall. However, there is usually a reasonable “tail” that spills over into longer wavelengths, which in practice makes some form of clean-up filtering essential. The available wavelengths, at least for sufficiently bright emitters, go all the way down to 365nm, which is tantalisingly close to the ideal requirement for fura2 of an excitation wavelength centred around 340nm. This can be made to work in practice, as the emission is sufficiently bright overall for there still to be a useful amount of light at those shorter wavelengths. Laser diodes have light to spare of course, and currently available (or should that be affordable?) ones go all the way down to 405nm. Again, there is a pretty good selection of wavelengths, albeit with an annoying gap in the green at around 560 nanometers, although laser-based alternatives are nevertheless available here.However, a much more attractive possibility for dealing with the lack of sufficiently short-wavelength sources has recently appeared which is instead to shift the spectral range of the fluorophore to rather longer wavelengths. This has already been done for calcium measurement, in the form of the fura8 analogue of fura2 (the fates of fura3 to fura7 remain sadly unknown), which has its entire excitation spectrum shifted to longer wavelengths by 20-30 nanometers. This type of development also has some very attractive implications for the microscope, specifically for the design of the objective – because “conventional” objectives begin to transmit light increasingly poorly at wavelengths below around 350-360 nanometers. That's been an important limitation because in the standard “epifluorescence” configuration the objective is also used to deliver light to the preparation, so must also be able to pass these wavelengths. Objectives that can function effectively at such wavelengths have a much narrower range of optical glasses to choose from for their design, for which the penalty is poorer chromatic correction (and of course higher price), so this type of development is good news all round, at least from the user point of view.
Optimising detection efficiency Now we come to the detection side of things, and here of course the goal is to make sure that as many of the photons as possible make it from the objective into your detector(s). Of course the objective needs to collect as many photons as possible, which means collecting over as wide an angular range as possible, which in turn means having as high a numerical aperture (NA) as possible. But it's all too easy to think that's all there is to it. It isn't. An awful lot of those precious photons can easily go astray without you realising, whether from losses that are mostly avoidable, or because they are in the system at times when they're not actually being used. Let's see what obstacles these photons may have to negotiate. The important point is that every optical surface that a photon encounters is a potential cause of its loss, and it there may be quite a number of them along the way. For example, the reflection efficiency of a standard aluminised mirror is about 95%. That may not sound so bad, but if there are say half a dozen of them in the pathway, their cumulative loss may be quite significant. Suddenly an ok-sounding 95% has become a far less acceptable 70% or so. Fortunately, there are “dielectric” coating alternatives, which offer reflection efficiencies of 99% or possibly even higher, but there is a potential penalty here in that such figures are generally achievable over a more limited range of wavelengths and/or reflection angles, so it is therefore very important that such mirrors are used within their design criteria, otherwise you could be losing your precious photons by the bucket-full.
The same goes for lenses, and perhaps even more so. For a start, each lens has two surfaces rather than just one, and here the typical loss from a bare surface (in this case by reflection rather than by the lack of it) is 4%. In fact, since many “lenses” may have multiple elements in order to successfully control optical aberrations, their very existence is only rendered feasible by surface coatings that substantially reduce these losses. Again, the same restriction applies – the better the performance within the design wavelength range, the worse it's likely to be outside it. This sort of consideration is well worth bearing in mind if you want to start exploring into the near infrared, which most modern cameras will allow you to do. And finally – although it's actually the first obstacle beyond the objective for our precious photons – we come to that strange but all-important component that is almost universally but incorrectly referred to as the “dichroic”. Dichroism is actually a polarisation-dependent optical phenomenon, but the nature of most biological fluorescence applications is that any polarisation dependency is effectively lost by virtue of the fluorophores having time to reorient during the period of their excited state, so that's something we generally don’t want. What we do want is an optical surface, positioned at 45 degrees to the light path, that reflects the excitation light (coming in from the side at 90 degrees) into the back of the objective, while transmitting the longer-wavelength emission light as efficiently as possible.This important component then requires some additional backup further along in the system, but often immediately after this mirror that blocks any remaining excitation light from reaching the detector(s). The performance of these two components, in respect of how efficiently they pass the wanted photons, while effectively blocking the unwanted ones, is clearly crucial for the good performance of the system as a whole. There is no point getting the most expensive camera that money can buy if photons are unnecessarily wasted here! This is an important practical point for two reasons. First, there have been recent improvements in optical filter technology that have given higher in-band transmission while retaining very high out-of-band blocking. Basically, these filters achieve their performance at what appears to be a single optical surface but is in fact a very carefully designed series of optical films of defined refractive index and thickness that have been deposited onto it. It's the interference effects from multiple internal reflections between these layers that gives these components (both the mirror and the filters) their required characteristics, so this technology is in fact very similar to that of the multilayer coatings that improve the optical efficiency of lenses and mirrors elsewhere in the system as already described. The second reason is that components designed this way are much more likely to retain their performance over time. The older technology, where filters in particular had a physical multilayer “sandwich” type of construction, is much more prone to deterioration over time, so it is well worth replacing any of those that your system may have.An awful lot of those precious photons can easily go astray without you realising, whether from losses that are mostly avoidable, or because they are in the system at times when they're not actually being used
[caption id="attachment_55455" align="alignnone" width="500"] Updating the amount of photons that can be caught will increase the quality of the pictures taken.[/caption]
We can also add, as a general point, that filter performances in terms of their spectral response have become increasingly better-matched to the emission properties of specific fluorophores in recent years, to transmit as many of the “useful” photons as possible. The goal here is not just to go for the peak, but to grab the whole lot. This is a further strong reason for updating your filter collection before you do anything else.
The detector system Only now is it worth directing your attention towards the detector system itself, which nowadays is typically one or more cameras. This is a subject in its own right of course, but typically a “good” camera is likely to have a detection efficiency on the order of 70% or so, with somewhat higher figures available for those with deeper pockets. Of course it would be nice to get to 100%, but again to make the point, one can so easily lose far more photons than that by giving insufficient attention to the rest of the system. We now come to that other potential improvement, namely of modulating the light source so that excitation light enters the system only when the detection system is able to receive it. This is an important point, because in practice one may not be providing just one excitation wavelength range and/or detecting just one emission wavelength range. This sort of situation also implies that the pathway to the detectors is likely to be more convoluted, which makes the foregoing discussion on optical losses correspondingly more relevant.
In particular, one may be using two or more fluorophores simultaneously, and/or using fluorophores whose excitation and/or emission spectra may change according their environment, allowing “ratiometric” detection methods to be employed. This is also a subject by itself, but if changes in an excitation spectrum are being monitored (again as for the calcium indicator fura2), then there is no alternative to applying the different excitation wavelengths sequentially. It's on the emission side where things get more interesting, and where there is better scope for using all the useful photons if you have the right equipment to do so. A simple example here is another calcium indicator, indo1. There is no significant excitation spectrum change here, so one can just continuously illuminate, in this case at wavelengths centred around 360 nanometers. When this indicator binds calcium ions we can follow this (and hence the calcium concentration change) by the increased fluorescence emission at wavelengths around 410 nanometers, and by the reduced emission at wavelengths around 485 nanometers. But how do we do this in practice?
The simplest possibility would appear to be to sequentially image through appropriate wavelength filters, but that's clearly wasteful because for every image you throw away all the photons that you could have used to obtain its partner. Also, filter changing is often done by some sort of filterwheel, which tends to be relatively slow and clunky at the best of times, so further photons are lost while you wait for the thing to move the appropriate filter into position – unless of course you are able to switch off the light source meanwhile. However, if your budget is up to it, you can use two cameras, with a wavelength-selective mirror to transmit light of one wavelength range to the first camera, and to reflect light of the other range to the second. But now your acquisition system has to deal with two cameras simultaneously, which may not be so easy or convenient. Therefore another alternative is frequently adopted, namely the use of an “image splitter”. This splits the light into two wavebands as before, but it projects two images onto different regions of the camera chip at the same time, saving you the cost of another camera and giving you just a single image for your acquisition system to deal with, and which can easily be separated into its individual components afterwards. This approach increasingly makes sense, because cameras with bigger sensors and correspondingly more pixels have become available in recent years, so in practice there may be no loss in spatial resolution as a result of only being able to use part of the sensor for each image.
In fact, we can take this approach even further, especially when dealing with multiple fluorophores, as splitters yielding three or even four images are commercially available, and systems using similarly larger numbers of dedicated cameras for each individual image can also readily be constructed. However, in multiple fluorophore systems there is an increasing risk that the excitation wavelength range for one overlaps the emission range for another, so crosstalk increasingly becomes a problem. That may require some sort of synchronised filterwheel to sort out, but even if we switch off the illumination while the wheel is changing position, we may be limited to taking just a few images per second because of the finite stepping time of the wheel. The ready availability of more intense light sources allows a much faster approach though. The wheel can now spin continuously, which is much faster than stepping it. Each filter will now be fully in the light path for only a short fraction of a rotation, but we counter that by a correspondingly more intense illumination for a shorter period. This allows much higher-speed imaging, but still with all those precious photons being fully utilised.Typically a “good” camera is likely to have a detection efficiency on the order of 70% or so, with somewhat higher figures available for those with deeper pockets
Dealing with the third dimension We now come to the elephant in the room, which is the third dimension. An “image”, as classically defined as something seen by a camera, is of course a two-dimensional construct, but most samples aren't going to be just surfaces; instead they will have depth. Yes, we can adjust the microscope so that some particular plane within the object is in focus, but the light from all the other depths will still be reaching our detectors. The problem can be addressed to some extent by image deconvolution techniques, where we obtain an entire “stack” of images at different focus depths, and then from what is in focus at any one depth we use to estimate it’s out of focus contributions at the others, allowing us to subtract them out. However, the process is computation intensive (although there have been recent improvements here), and the subtractive process is not ideal, as optical signals are inherently noisy, and when one subtracts signals the noise actually tends to add. Clearly it would be better to somehow look at only “one” depth at a time, and this is where confocal techniques come in. These generally work by some sort of “scanning” principle, where one or a series of sufficiently widely spaced points in the object are illuminated at a time. Only at the focus depth of interest will the illumination approximate to a point; at other depths it will increasingly spread out, giving a 3D structure of two opposed cones with their apexes touching at the depth of best focus. The trick is then to de-scan the image so that as the illumination points are moved around in order to eventually illuminate the entire sample, the emission light has to pass through one or a series of small apertures that move around in tandem (hence “confocal”) with the illumination points. Only at the depth of best focus can the emission light efficiently pass through these apertures, because of that cone-shaped illumination profile, so we have nicely solved our problem.
But wait – we've just thrown away a huge proportion of those precious emission photons! From the goal we set ourselves, of minimising “photons in” and maximising “photons out” isn't that the last thing we want to do? One possible mitigator here is also to harvest some or all of those rejected photons, as they do contain potentially useful information of course, but that can only be a compromise at best. Wouldn't it be much better only to illuminate at the depth of interest?Clearly it would be better to somehow look at only “one” depth at a time, and this is where confocal techniques come in
Two-photon or lightsheet? Actually there is a very clever way to do this, but its adoption has been hindered by a variety of practical difficulties. This is the “two-photon” technique, where a fluorophore can effectively be tricked into almost simultaneously absorbing two photons of half the required excitation energy (hence double the wavelength), but with the same effect as that of a single photon of twice that energy. We still get that cone-shaped illumination profile through the sample, but only at their joint apex is the illumination intensity high enough for this to occur to any significant extent, because the two-photon effect is, by definition, a square-law. This can and does work, but it's probably fair to say that the practical limitations have been greater than expected, otherwise everybody would be using it by now. The main problem is that the illumination intensities required for two-photon absorption are truly enormous, and they must be achieved only for very short periods, typically less than a picosecond. This requires the use of expensive pulsed lasers that require careful alignment, and there are also safety implications in that one is dealing with very intense radiation at wavelengths that you cannot see (or to put it more brutally, that you would only ever see once). Furthermore, the fluorophores aren't completely fooled, so their behaviour under two-photon excitation isn't exactly equivalent to that of the single-photon case. So, to get the best out of the technique, one really needs fluorophores that have been more specifically engineered for two-photon excitation. That's not easy, although some progress is being made. However, the sad bottom line is that this technique has only partially delivered on its potential theoretical advantages, so more recently people have been looking at other ways to tackle the problem.
This brings us to the rapidly-developing area of light-sheet microscopy. It takes many forms, but the basic idea is to illuminate from the side, so that fluorophores only over a limited range of depths are excited. There are both theoretical and practical difficulties here too though. The theoretical one is that a sheet of light can be made really thin over only a limited length, although it's certainly enough to help, and on the practical side there may be difficulties in incorporating your sample into a system configured in this way. In general, it's most suited to relatively large samples, which are deep enough for the relatively narrow depth range of illlumination to be really useful in practice. However, perhaps the most exciting feature of this technique is that it's sufficiently simple and straightforward to be used by anyone, so we'll certainly be hearing much more about it in the future.A new form of fluorescence microscopy is currently turning scientists' heads - light sheet microscopy.
Nonlinear photobleaching effects Finally, we come to the very interesting topic as to how the illumination of our fluorophores is best distributed over time. We already have our cardinal rule of switching the light source on only when our detection system is ready, but is there more to it than that? Well, it turns out that there is, although there is still much uncertainty as to details. The first clue here was the experimental observation that confocal systems based on single-point scanning appeared to cause more photobleaching than multipoint ones where the apertures are on spinning discs, for example. If you have more scanning points for a given overall photon dose, then the instantaneous dose from one aperture out of many is going to be correspondingly lower than in the single-point case. A possible theoretical model for this sort of effect is the driving of the fluorophores into a triplet state. At each excitation, the probability of a fluorophore converting to this state rather than undergoing fluorescence emission is low, but this state has a much longer lifetime, so at higher excitation intensities the proportion of fluorophores in this state will be higher. If one now proposes that absorption of a second photon by a fluorophore in this state would bring about its destruction (very likely in view of the doubled energy), then we have a very reasonable model for what may be going on here. It's another type of two-photon effect, although not a desirable one in this case, but giving a similar square-law type of behaviour.
Clearly, at these intensities there are advantages in delivering the excitation photons on a more rather than less continuous basis, but where exactly is the threshold? That's likely to be a difficult question, especially if the above model is correct, because it will then vary according to the characteristics of the individual fluorophore. More recently though, there have been some suggestions in the literature that, albeit over lower intensity ranges and longer timescales than these, for the opposite effect – namely that discontinuous illumination is somehow “better” than continuous. However, it's not clear to this author whether the effect is just because of a lower overall photon dose from the light source being not being on all the time, or whether something more interesting is going on. We at Cairn currently suspect the former, but why theorise when you can do the experiments? In collaboration with one of our Royal Society Visiting Research Fellows, Dr Dan Mulvihill from the University of Kent at Canterbury, we've just started to have a look for ourselves. Dan has a very nice model system based on yeast, on which we can investigate both photobleaching and phototoxicty.Let's see what we can find out!
Author
Dr Martin Thomas is President of Cairn Research Ltd. www.cairn-research.co.uk