Are we close to the stem cell revolution?
4 May 2016 by Evoluted New Media
Stem cell therapy has been in use for many years, but with only limited reach. As such the oft bandied stem cell revolution has still yet to arrive. Steve Buckwell and Chris Coe explain why this is set to change and why now is the perfect time for its potential to be achieved.
Stem cell therapy has been in use for many years, but with only limited reach. As such the oft bandied stem cell revolution has still yet to arrive. Steve Buckwell and Chris Coe explain why this is set to change and why now is the perfect time for its potential to be achieved.
The stem cell revolution as it's often referred to is now already in its third decade. But like the paper free office, is it just one of those envisaged futures that never seem to really happen? Embryonic stem cells were first isolated 18 years ago, but stem cell therapies have been slowed by high production costs, batch-to-batch variability and limited seed material. But we still believe the revolution will kick off some time in the second half of this decade. This is why.
Firstly the early ethical issues have, in many cases been overcome, with adult stem cells showing promise in the clinic but not requiring the embryo exploitation and destruction that made embryonic stem cell research so controversial in the years after 1998. Secondly, there is now substantial mid-stage clinical evidence that stem cells work in areas of unmet medical need, much of which has only become evident in the last five years.There are various stem cell products in development that work allogeneically, meaning that the patient receives stem cells sourced from someone else’s body. As a general rule, allogeneic therapies are quite cost effective because they have the potential to be ‘off-the-shelf’, whereas autologous therapies (use of the patient’s own cells) can be considerably more expensive.
It has only recently been proven that stem cells can be made at industrial scale whilst conforming to Good Manufacturing Practice, the guidelines regulators require drug makers to abide by. It is this latter, the scale up from the laboratory to an 'off the self' product which has the capacity to slow the train down. After all, a product that can't be manufactured isn't a commercial product – it's an academic curiosity. Many interesting molecules sit on the shelf in pharmaceutical companies waiting for solutions to the manufacturing challenge. Fleming discovered penicillin in 1928, but it took a World War, countless dollars and the combined brains of innumerable world class institutions to develop a working product. Initially we'll take a look at the way stem cells are generated and expanded. This can be broken down into two areas; those produced using embryos in one form or another, and those that do not.
Back in 1998 in a stem cell ‘Eureka moment’, James Thomson and colleagues at the University of Wisconsin generated the first human embryonic stem cells (hESCs) using tissue from embryos fertilised in vitro. This method uses embryos that have been generated during IVF and are no longer required. However, hESC lines are extremely difficult to grow in culture; the cells require highly specialised growth media that contain essential ingredients that are difficult to standardise. Yet the culture conditions are critical to maintain the cells’ self-renewing and pluripotent properties, so therefore hESCs do not lend themselves to mass production.
[caption id="attachment_53477" align="alignnone" width="430"]It has only recently been proven that stem cells can be made at industrial scale whilst conforming to Good Manufacturing Practice
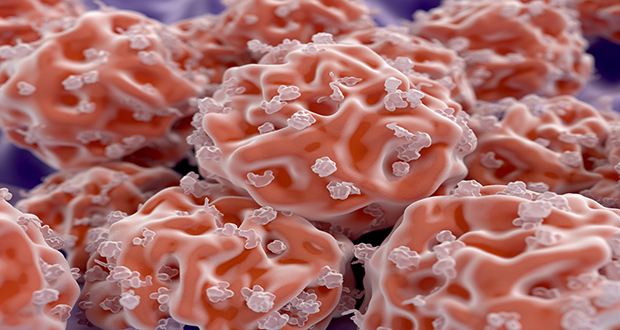
A second method for generating human pluripotent stem cell lines was published in 1998 by John Gearhart and co-workers at The Johns Hopkins Medical School. These researchers isolated specialised cells known as primordial germ cells (PGCs) from a five to seven week old embryo and placed these cells into culture. As with hESCs, PGCs present challenges with sustained growth in culture. Spontaneous differentiation, which hinders the isolation of pure clonal lines, is a particular issue, meaning once again this is unsuitable for scaling up. Embryos that stop dividing after being fertilised in vitro are not preferentially selected for implantation in a woman undergoing fertility treatment. These embryos are typically either frozen for future use or discarded. In 2006, scientists generated hESC lines from IVF embryos that had stopped dividing. These scientists used similar methods as described for traditional hESC line generation but with an exception – their source material was so-called “dead” IVF embryos. In one sense this method might be seen by many as a more ethical route. The human stem cells created using this technique behaved like pluripotent stem cells, including producing proteins critical for “stemness” and being able to produce cells from all three germ layers. Several other embryonic based methods of production exist, such as hESC lines from genetically abnormal embryos, from single cell embryo biopsy and those created via parthenogenesis. But again these were never going to be practical routes to the scale up challenge.
The promising area is unquestionably the creation of truly pluripotent stem cells from non-embryonic routes. When you think about it the only practical alternative to searching for an existing population of stem cells is to create a new one from a population of non-pluripotent cells. This strategy, which may or may not involve the creation of an embryo, is known as “reprogramming.” Current approaches to this include reprogramming through somatic cell transfer, cell fusion or altered nuclear transfer.In somatic cell nuclear transfer (SCNT) human oocytes (eggs) are collected from a volunteer donor who has taken drugs that stimulate the production of more than one oocyte during the menstrual cycle. Scientists then remove the nucleus from the donated oocyte and replace it with the nucleus from a somatic cell, a differentiated adult cell from elsewhere in the body. The oocyte with the newly transferred nucleus is then stimulated to develop. The oocyte may develop only if the transplanted nucleus is returned to the pluripotent state by factors present in the oocyte cytoplasm. This alteration in the state of the mature nucleus is called nuclear reprogramming. When development progresses to the blastocyst stage, the inner cell mass (ICM) is removed and placed into culture in an attempt to establish a pluripotent stem cell line. To date, the technique has been successfully demonstrated in two primates.
There is also reprogramming though cell fusion. In 2005 researchers at Harvard University reported that they had fused cultured adult human skin cells with hESCs. The resulting “hybrid” cells featured many characteristics of hESCs, including a similar manner of growth and division and the manufacture of proteins typically produced by hESCs. Some factor(s) within the hESCs enabled them to “reprogram” the adult skin cells to behave as hESCs. However, these cells raised a significant technical barrier to clinical use, fused cells are tetraploid (they contain four copies of cellular DNA rather than the normal two copies), and scientists would need to develop a method to remove the extra DNA without eliminating their hESC-like properties. The fusion method serves as a useful model system for studying how stem cells “reprogram” adult cells to have properties of pluripotent cells. However, if the reprogramming technique could be carried out without the fusion strategy, a powerful avenue for creating patient-specific stem cells without using humans could be developed.Lastly there is reprogramming through altered nuclear transfer (ANT). This is a variation on standard SCNT that proposes to create patient-specific stem cells without destroying an embryo. In ANT, scientists turn off a gene - CDX2 - needed for implantation in the uterus in the patient cell nucleus before it is transferred into the donor egg. However, the real potential for mass production of stem cells lies with induced pluripotent stem cells (iPSCs). This is where true scalability starts to get exciting, and involves the use of identities known as mesenchymal stem cells (MSCs). MSCs are multipotent stromal cells that can differentiate into a variety of cell types. Existing methods of large scale MSC production rely on the isolation of these cells from donor tissue followed by expansion, or harvesting as it's sometimes called. The quantity of cells produced in this way can in theory proceed by almost exponential growth, so it would seem to be suitable for industrial scale production.Pluripotent stem cells provide a limitless starting material, derived from essentially any tissue in the body, and there are now over 300 clinical trials underway with MSC therapies
However, there are difficulties with this approach since the MSCs grown start to lose potency quite quickly and ultimately stop dividing altogether in a process defined as senescence. In addition the number of cells that can be isolated from each donation is quite limited, typically being of the order of 2x104 MSCs per donation, whereas a clinical dose of around 108 MSCs would be the average requirement. With this in mind, a continuous supply of new donors would then be required and this in turn inevitably adds greatly to the cost of the therapy. Donation procedures can be both invasive and painful, especially where bone marrow is the tissue involved. Then, of course screening procedures can be time consuming. Finally there is the issue of consistency of end product. Clearly this is very difficult to achieve when relying on multiple donors for supply and so this approach therefore does not lend itself to commercial deployment.
An approach to overcome these problems is based upon the work of Professors Slukvin and Thomson et al in the generation of induced Pluripotent Stem Cells (iPSCs) – and in the discovery of Mesenchymal angioblasts (MSAs). IPSCs are essentially a man-made version of hESCs, derived from adult cells and have very similar characteristics to hESCs. They also avoid the ethical concerns, since they are not derived from human embryos. These two scientists were pioneers in the development of iPSCs and were one of two independent research groups that first reported the creation of iPSCs from human cells. Critically, iPSCs - like ESCs, can be expanded without limit, be stored over long periods and produce tissue cells of any type. This of course makes iPSCs an ideal building-block for cell-based therapies.
IPSCs themselves are not administered directly to patients as medical treatments. Instead, they are used as a starting material to produce other types of cells, which may have the potential to be used as therapeutic agents. Once these advances in research were made, it was suggested that similar techniques might be used to reprogram adult human cells. In 2007, Yamanaka et al reported that introducing the same four genetic factors that reprogrammed mouse cells into adult human dermal fibroblasts reprogrammed the cells into human iPSCs. These iPSCs were similar to hESCs in numerous ways. Moreover, the cells could differentiate into cell types from the three embryonic germ layers.
[caption id="attachment_53476" align="alignnone" width="430"] Induced pluripotent stem cells: similar to embryonic stem cells but made from adult specialised cells using a laboratory technique discovered in 2006.[/caption]
The cells generated by the Thomson group met all defining criteria for hESCs, with the exception that they were not derived from embryos. The search was therefore on for precursors of MSCs – and that is where mesenchymal angioblasts come in. MCAs are an extremely important class of early clonal mesendodermal precursor cells that evolve into both MSCs and endothelial cells. Later studies by the Wisconsin group identified a common precursor of mesenchymal and endothelial cells, mesenchyme angioblasts, as the source of mesoderm-derived MSCs. In other words an exciting route to generation of MSCs had been identified – and that has led to the following route to be identified and now verified at the Wisconsin based Madison Group. So commercialisation is at last becoming a reality.
Pluripotent stem cells provide a limitless starting material, derived from essentially any tissue in the body, (although blood cells are the most commonly used), and there are now over 300 clinical trials underway with MSC therapies. A great deal of progress and hard work in this area has already been achieved by companies like Athersys and Mesoblast, which have identified the potential utility of MSCs. One technology belonging to Cynata Therapeutics Ltd and known as Cymerus combines iPSCs with MCAs and was recently validated at a US bio-manufacturing plant under Good Manufacturing Practice. Critically the Cymerus process can source all the cells ever needed from a single donor, thus eliminating product variability and the need to constantly seek out new donors. Watch this space. The industry's ability to generate robust, consistent and inexpensive cells is set to accelerate the commercialisation of stem cell therapies worldwide.
Authors:
Steve Buckwell has a PhD in physical organic chemistry and worked in pharmaceutical research until starting his own business in the 1990s.Chris Coe has a varied background in marketing, business development and corporate finance. He has collaborated with Dr Buckwell on a number of projects.