Matter over mind
10 Sep 2019
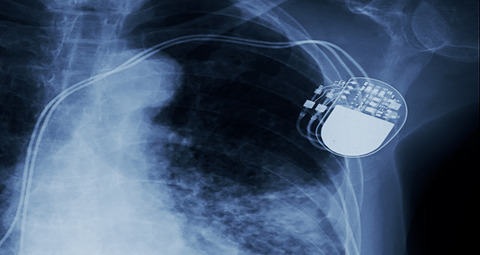
Materials science and nanotech are vital for developing implantable microdevices but there are several key issues to overcome before a device can be successfully used to tackle neurological disease. Here Dr Hadi Heidari and Dr Rupam Das take us through the challenges…
Developing microscale brain implant devices pursues the futuristic concept of curing disabling brain complaints by means of brain tissue transplants. It offers a certainty that so far has only available for other human body organs.
Neurological Diseases such as epilepsy, dementia, Alzheimer’s and Parkinson’s Diseases affect 67% of Europe’s population. To date, epilepsy affects 50 million people worldwide, 8 million of whom live in Europe, this comes with an enormous financial commitment to deal with these diseases –approaching €336 billion per year. Moreover, neurological disorders affect 58% of people living in developing countries. This figure is expected to rise to 71% by 2050.
In the quest for novel methodologies to defeat brain disorders, research is investigating several strategies. Emerging methodologies are based on regenerative medicine (tissue engineering) or on neural engineering as distinct approaches. However, inherent drawbacks limit their sole exploitation.
When biomaterials are designed, a set of properties are built in such a way to ensure that, after implantation, they will help the body to heal itself
Regenerative medicine aims at repairing anatomical brain damage, but whereas the intrinsic neuronal plasticity of biological grafts offers an exceptional degree of flexibility, their unpredictable behaviour might endanger the patients’ safety unless adequately fine-tuned. On the other hand, neural engineering proposes to replace brain function via highly controllable brain-inspired systems; yet, the flexibility of these tools is still far from brain performance and comes at the cost of the inability of rebuilding brain matter.
Integrating neural engineering and tissue engineering requires a compliant and stable interface between the rigid device and soft tissue, that can be challenging. Exploiting biocompatible offers an attractive solution. The development of such imperceptible materials also provides the intriguing possibility of expanding the capabilities of human brain health.
Biocompatibility, flexibility and biointegration
There are specific requirements that an implant or device must meet for long-term use in an in-vivo environment. If any of these requirements are not satisfied, the user may experience side effects or even death. The characteristics of an optimal brain implant include ease of insertion, mechanical flexibility, excellent biocompatibility, suitable biointegration, predictability of long-term behaviour, and affordability.
Biocompatibility
From the biological perspective, materials used in devices and substrates for implants must be composed of materials that are bioinert depending on the requirements, and biocompatible with respect to their ability to demonstrate appropriate responses in specific situations. However, this characteristic depends on the type of material used. One challenge in the selection of biomaterials for use in these implantable devices is the consideration of the areas in which the materials are being positioned within the body.
Different systems within the body contain different kinds of chemicals, have different pH levels, and require different mechanical parameters. Therefore, a material used for a device in one region of the body may not be used for another device in a different region of the body. This makes the determination of a material for general purposes difficult, and characterization can only be conducted according to specific devices and purposes. As most neurological diseases are chronic in the brain, therefore, use of a suitable biocompatible material for encapsulation as well as proper device modelling is critical for the long-term implantation of the brain implantable devices.
In general, biocompatible materials can be ceramics, metals, polymers, and polymer composites. Ceramics show good biocompatibility, corrosion resistance, and high compression resistance and density. Some disadvantages of ceramics include brittleness, low fracture strength, low mechanical reliability, lack of resilience, and relatively difficult fabrication. Metals have high strength, density, ductility and resistance to wear. In contrast, polymers are available in a wide variety of compositions, properties and forms. They can also be fabricated readily into complex shapes and structures. When multiple types of polymers are used in cohesion in one thin layer, such as in a polymer composite, the properties of the layer differ compared to their parent materials. However, the composite material simultaneously has a low elastic modulus and high strength with a greater potential for structural biocompatibility compared to the separate parent materials. Corrosion and fatigue failure of metal alloys do not occur, and the release of metal ions is prevented, and fracture toughness is increased as compared to ceramic materials. Polymer and their composites benefit from further advantages such they are nonmagnetic and radio transparent for X-ray radiography and magnetic resonance imaging (MRI) scans.
Biocompatible polymers such as Parylene C, PDMS (Polydimethylsiloxane), Polyimide, BCB (Benzocyclobutene) are most commonly used for chronic implantation. In addition, these materials are a better match mechanically to the tissue, and deforms as the tissue moves, flexible neural probes made of these materials can prevent the gradual decrease in the signal-to-noise ratio.
Biointegration
When biomaterials are designed, a set of properties are built in such a way to ensure that, after implantation, they will help the body to heal itself. Therefore, it is critical that these materials be integrated into organ specific repair mechanisms. It should involve a direct structural and functionally stable connection between the living part and the surface of an implant. Although various materials have been developed in recent years with enhanced physical, surface and mechanical properties, the use of these materials in certain biological applications is often limited by poor tissue integration. So, the question is on how biomaterials can be converted to ‘living tissues’ after implantation.
Emerging nanobiotechnology is revolutionizing our capability to resolve biological and medical problems by developing subtle biomimetic techniques. The use of nanoscale materials is expected to increase dramatically in many applications of medicine and surgery. It is interesting to note that the size of these nanomaterials is comparable to many biological systems and so there is large scope for biointegration. Additionally, nanomaterials exhibit fundamentally different properties from their properties in bulk, such that they can be tailor-made to provide properties to suit a specific application. Significant advancements are expected to achieve the desired goals and their clinical use, especially in areas such as nanostructured coatings and other nanobiomaterials. The current trends in nanobiotechnology, thus, offer a bright future through the use of nanobiomaterial in achieving biointegration.
Mechanical flexibility
An implantable device which includes sensing and electronics components should be properly packaged or encapsulated before in-vivo installation. The encapsulation acts as a protective layer preventing the movement of waste materials between both the device and the human. To enable a foreign object to be implanted into the body of a human, size matters not only during the implantation procedure, but also for the entire duration that the object remains in the body. The size also determines the survivability of the object and the comfort of the user. Thus, the object must be compact to reduce the stress on its surrounding tissues, muscles, and bones where the object has been implanted. The small size of the object also allows minimally invasive procedures to install these devices. Although the reduced size may decrease the structural integrity of more delicate devices, the demand for comfort often outweighs concerns about structural integrity. Although neural probes can be shaped precisely and have sufficient strength to penetrate neural tissue, the mechanical mismatch between these stiff substrates and soft tissue aggravates micromotion at the tissue–electrode contact sites, which induces inflammation in the tissue.
Furthermore, the encapsulation must also be able to withstand stresses and shocks, as the human body is in a constant state of motion, and the occasional high and sudden impulses resulting from body exercises and sudden motions. The encapsulation must be flexible enough to endure these forces when the implants are used as additional support or infrastructure for the body to carry out its own regeneration and healing. Additionally, because the implants and devices are constantly experiencing thermal influence from the human body, the encapsulation must be able to perform its function at body temperature for the required amount of time. In summary, our research bridges the tissue engineering and neural engineering by making use of biocompatible, flexible materials to design the neural electrodes as well as for device encapsulation.
The Microelectronics Lab
The Microelectronics Lab (meLAB), is part of the FET-PROACTIVE Hybrid Enhanced Regenerative Medicine System (HERMES) project supported by the European Commission’s Future and Emerging Technologies. This €8.4M project is among the most ambitious and competitive in Europe and is coordinated by Istituto Italiano di Tecnologia (IIT) in Italy and involves 12 partners from Italy, Spain, Denmark, United Kingdom, Finland, Belgium, and The Netherlands. In the UK, researchers from the University of Glasgow will work to make the microelectronic chip implantable into the brain.
meLAB aims to promote and support engineering and physical science research in microelectronics design, spintronics, magnetic sensors, and energy harvesting. The research is broadly ranging from theoretical, simulation, design, fabrication, and experimental work in fundamental physics to applications of wearable and implantable electronics.
Authors:
Dr Hadi Heidari is Assistant Professor based in the Microelectronics Lab (meLAB) at the University of Glasgow
Dr Rupam Das is a Postdoctoral Research Associate at meLAB