Law and disorder
23 Sep 2019
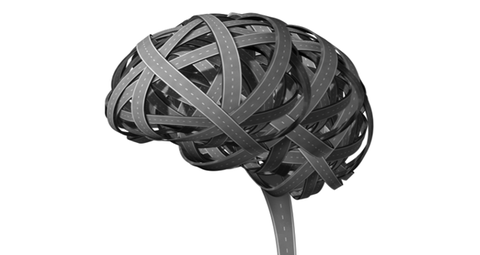
We have been working on Alzheimer’s for a long time… so where are all the therapeutic drugs? Here Dr Sean Devenish looks at the biggest challenge for developing an effective therapy and wonders if there is a light at the end of the tunnel in building our biological understanding of the disease while also improving the drug development pipeline...
Since 1998 there have been 146 failed drugs in the search for a treatment against Alzheimer’s disease. In this same period, just four drugs have been brought to market1. But why is the success rate for Alzheimer’s disease drug trials so low?
For drugs that target other therapy areas, the likelihood of a drug being brought from phase one trials through to market is 15.3%, however, for Alzheimer’s drugs this drops to 7.3%. But with nearly 10 million new cases every year – that’s one every 3 seconds – dementia is already a public health priority, and with cases set to triple and reach 152 million by 20502, drugs to treat Alzheimer’s disease are critical.
Protein misfolding, amyloid, and disease
Genes in DNA encode protein molecules, which fold into precise three-dimensional, soluble structures capable of performing a specific task and function within a human cell. This central dogma is taught to budding scientists the world over but protein misfolding breaks these laws and challenges this understanding.
Figure 1: Since 1998 there have been 146 failed drugs and only 4 successful drugs in the search for a treatment against Alzheimer’s disease
Misfolding of normally soluble peptides and proteins converts functional molecules into aggregates such as fibrils, which not only exhibit a loss of function but, in the case of fibrils, often also generate toxic intermediates during self-assembly. Amyloid fibrils are a key example of this, and are strongly associated with ageing disorders including Alzheimer’s disease.
While it represents one of the most researched diseases around the globe, with the NIH expected to spend $2.3 billion on Alzheimer's research in 2019, the molecular mechanics of Alzheimer’s disease remain poorly understood in comparison to other diseases
Amyloid fibril plaques found in the brains of patients with Alzheimer’s disease are mainly composed of a peptide called amyloid beta (Aβ). These peptides contain approximately 40 amino acids and are derived from a protein called amyloid precursor protein (APP), which can be cleaved by beta secretase and gamma secretase to generate Aβ peptide. While the normal biological function of Aβ is not well understood, its role in Alzheimer’s disease pathology is intensely studied but also remains challenging to fully understand.
Biophysical studies are helping to reveal important molecular details involved in the formation of fibrillar aggregates, whereby the aggregation process consists of a complex cascade of individual steps of nucleation and growth. Figure 2 highlights some of these key processes and shows how monomers can undergo primary nucleation to create fibrils. This is followed by a process of elongation and then an especially critical step of secondary nucleation, which increases the rate of formation of low molecular weight oligomers. These accompany the formation of mature fibrils but are also thought to represent the most toxic species for neuronal death and Alzheimer’s pathology. Being able to control these key aggregation processes with specific inhibitors represents an attractive therapeutic opportunity to combat amyloid associated diseases such as Alzheimer’s.
Figure 2: Schematic representation of the formation of fibrillar aggregates
The mechanics of Alzheimer’s
While it represents one of the most researched diseases around the globe, with the NIH expected to spend $2.3 billion on Alzheimer's research in 20193, the molecular mechanics of Alzheimer’s disease remain poorly understood in comparison to other diseases. A small proportion of Alzheimer’s cases can be linked to inherited genetic differences but for the majority of patients, the cause remains mostly unknown. As such, research into potential treatments to stop or reverse disease progression continues to be challenging. Though some therapies are available to temporarily improve symptoms, a cure remains elusive.
One well-known hypothesis for Alzheimer’s identifies extracellular deposition of Aβ – as amyloid plaques and toxic oligomers – as the fundamental cause of disease4. However, a number of recent high-profile clinical trials for therapeutics that target this mechanism have failed and shown no positive clinical effect, demonstrating that there is still much we do not understand about the molecular mechanics of Alzheimer’s disease pathology.
The disordered and aggregated nature of the proteins involved mean that they are particularly challenging to study. Professor Sara Linse from Lund University explains: “Everyone told me not to touch amyloid beta since it is renowned to be difficult to study. They told me it is the peptide from hell; I’d never succeed.” But complex, challenging proteins such as Aβ are implicated in many important diseases and conditions, and many are emerging as interesting therapeutic targets. In fact, 20-30% of human proteins are considered disordered or have significant portions of disorder within them.
Because protein aggregates are highly heterogeneous and dynamic, conventional methods for studying protein structure and function are often not suitable or sufficient. This is particularly true when investigating protein interactions, which are crucial for elucidating molecular mechanisms of disease and in drug discovery when designing therapies to interfere with specific pathways and events. Traditional methods like surface plasmon resonance (SPR) – often the de facto method for studying protein interactions – require a protein to be attached or embedded to a surface. For proteins or peptides with a high level or disorder such as Aβ or for aggregates like amyloid fibrils, this immobilization is difficult to carry out and often results in unreliable results as it doesn’t represent the natural state.
Studying challenging proteins: The future?
Being able to study protein interactions in-solution, in their native environments and without having to immobilize the molecules onto a surface, increases the potential targets that can be investigated. Complex targets and aggregated proteins that can be challenging to attach to surfaces or embed in gels can be studied, as well as disordered proteins that do not have defined globular structures and therefore are susceptible to perturbations when attached to surfaces. In addition, looking at proteins in their near-native environments can produce more realistic and true-to-life results, giving researchers confidence in their findings.
Microfluidic diffusional sizing (MDS) is an innovative method of protein analysis developed by a spinout from the University of Cambridge (Figure 3). The method can be used to assess on-target protein interactions in solution and in crude biological backgrounds – opening up the possibilities for detailed analysis of proteins in their near-native states and natural environments. This has implications both for research – to understand more about the cause of Alzheimer’s disease – and also during drug discovery when designing potential therapies. By utilising native-state methods to measure protein-compound interactions, fewer drugs will fail in the later stages of clinical trials, which saves millions in drug research.
Figure 3: The Fluidity One-W measures the rate of diffusion of proteins under steady state laminar flow in a microfluidic chip - a technique known as microfluidic diffusional sizing (MDS). A stream of fluorescently labelled protein is introduced alongside an auxiliary stream. These streams flow in parallel and because there is no convective mixing, the only way the protein of interest can migrate into the auxiliary stream is by diffusion - the rate of which will depend on the size of the protein. Small proteins will diffuse very rapidly, and large proteins and aggregates more slowly. At the end, the streams are re-split, and at this point the degree of diffusion is fixed. The quantity of protein in each stream is then determined by the fluorescence from the label. The ratio of the fluorescence between the two streams gives us the protein's hydrodynamic radius (Rh).
The diffusional sizing method has been applied to study some of the key proteins implicated in Alzheimer’s disease to dissect the interactions and detailed molecular mechanisms underpinning the disease. During the FEBS/EMBO Women in Science Award plenary lecture at FEBS 2019 in Poland, Professor Linse presented work on amyloid proteins and key insights for novel therapeutic approaches in Alzheimer’s disease. She expalins, “With diffusional sizing, we were able to confidently generate accurate and complete in-solution data using amyloid proteins to connect stoichiometry and binding affinities with protein self-assembly.”
A recent paper in Science Advances5 also demonstrates the power of diffusional sizing for probing protein aggregates. The study looked at naturally occurring molecules that aim to protect against the deleterious effects of protein aggregates, molecular chaperones. The researchers used diffusional sizing to better understand how these molecular chaperones recognize and bind to Aβ protein aggregates. They were able to generate quantitative data including binding constants and kinetics, which has previously been very challenging using conventional methods. Two chaperones were studied, the Brichos domain that suppresses secondary nucleation processes and clusterin, which the researchers determined is capable of specifically inhibiting the elongation of Aβ fibrils by interacting with fibril ends at high affinity. This work also showed that the two different inhibitory effects of these molecular chaperones are additive and noncooperative, indicating that the reactive sites associated with the formation of new aggregates – through secondary nucleation – and the growth of existing aggregates – through elongation – are distinct.
This work clearly demonstrates the intricacies and subtleties surrounding the complex nature of Alzheimer’s disease pathology. New technologies that enable the elucidation of precise molecular details – such as the interplay between two molecular chaperones involved in aggregate formation and inhibition – help researchers to piece together the bigger picture. Only by unravelling the complex network of protein interactions and pathology in Alzheimer’s disease, can targeted treatments be developed that stand a chance of succeeding at clinical trials and beating the odds to be brought to market.
References
- PhRMA analysis of Adis R&D Insight Database, 25 January 2018 4. PhRMA analysis of Adis R&D Insight Database, 25 January 2018 5. Cummings, J et al (2014). Alzheimer’s disease drug-development pipeline: few candidates, frequent failures Alzheimer’s Research & Therapy 2014 6:37
- https://www.who.int/mental_health/neurology/dementia/infographic_dementia.pdf?ua=1
- https://report.nih.gov/categorical_spending.aspx
- Hardy, J. et al. Amyloid deposition as the central event in the aetiology of Alzheimer's disease. Trends in Pharmacological Sciences, 12 (10): 383-88
- Scheidt, T. et al. Secondary nucleation and elongation occur at different sites on Alzheimer’s amyloid-β aggregates. Science Advances, 5 (2019)
Author: Dr Sean Devenish is Head of R&D at Fluidic Analytics Ltd